Protein Folding and Aggregation, Mentoring, and a Molecular Food Recipe
Anna Mitraki
Professor at the Department of Materials Science and Technology
University of Crete
and affiliated Research Scientist at IESL, FORTH
Greece
mitraki@materials.uoc.gr
Alexander the Great in a historical statement, said for his Mentor, Aristoteles, “To my parents I owe my life, to my Mentors I owe the quality of life”. While «ΖΗΝ» (for life, to live) is easy to translate, “Quality of life” is the best phrasing that I found for translating «ΕΥ ΖΗΝ», that literally means the “GOOD LIFE” but is much more than that….
In my scientific path, I was very fortunate to have two great Mentors: my doctoral advisor, Prof. Jeannine Yon-Kahn, of Université Paris-Sud, France, and my post-doctoral advisor, Prof. Jonathan King of MIT. Under their mentorship, I worked on the scientific problem of “Protein folding and aggregation”. To put it in lay terms, trying to decipher the mechanisms that determine the structure and biological function of a very important class of biological molecules, i.e., proteins. When this process goes wrong, proteins misfold, fail to reach their native state and form aggregates (a process analogous to the formation of coagulates from boiled egg whites). It is this part of “protein misfolding” that forms the unifying theme of my scientific path and the most important part took place at the King lab.
Coagulation of a biological material upon heating was a characteristic diagnostic feature of the proteinaceous character. British biochemists M.L. Anson and A.E. Mirsky in a 1931 article entitled “The reversibility of protein coagulation” (Anson 1931) pointed that “Protein coagulation, together with combustion and fermentation, was one of the first chemical processes recognized by man”. The X-ray diffraction pattern of stretched, boiled egg white strips was recorded by W.T. Astbury and colleagues (Astbury 1959). The pattern displayed two characteristic reflections, one at 4.65 Å on the meridian assigned to “backbone spacing” and one at 9.8 Å on the equator assigned to “side chain spacing”. This particular pattern implied that the albumin polypeptide chains in the coagulated state were lying perpendicular to the axis of stretching in an extended beta-structure arrangement, termed “cross-beta”.
Τhis was considered a “useless” process at the time that was just generating waste material, and very few scientists were willing to address it. From both Mentors I acquired the taste of recognizing the need to tackle this kind of problem despite the associated difficulties. In the context of my thesis, I worked on elucidating the formation of aggregates during the unfolding /refolding pathway of a glycolysis enzyme, horse muscle phosphoglycerate kinase. In intermediate concentrations of denaturants, aggregates were forming. Following incubation under equilibrium conditions and subsequent transfer to renaturing conditions, in the range of these intermediate concentrations, enzymatic activity does not return, in contrast to activity return following completely denaturing conditions and subsequent transfer to renaturing conditions (Mitraki et al. 1987). This phenomenon was first recognized by Prof. Michel Goldberg of Pasteur Institute in Paris, that insightfully attributed it to aggregation of partially folded intermediate states. Namely, a model was proposed that involves protein segments that get exposed upon partial unfolding and associate with their complementary segments belonging to other molecules through native-like, but still incorrect, intermolecular associations (London, Skrzynia, and Goldberg 1974).
The year that I arrived at the King Lab, my colleague and subsequently lifelong friend, Cameron Haase-Pettingell, published a landmark paper entitled “Formation of aggregates from a thermolabile in vivo folding intermediate in P22 tailspike maturation – a model for inclusion body formation” (Haase-Pettingell and King 1988 ). The intellectual connection of the similar nature and mechanisms of formation between the in vivo and in vitro aggregation was then made. Inclusion bodies derive from partially folded intermediates and not from fully unfolded, or native forms of proteins. A review entitled “Protein folding intermediates and inclusion body formation” explicitly laid out the distinct nature of protein aggregates and the specificity in their formation pathways (Mitraki and King 1989). The specificity of interactions between folding intermediates implied that they were not amorphous tangles of material, but retained “quasi-native” structural elements (Figure 1). The specificity of interactions was elegantly demonstrated and mapped by the King Lab over the years through the effects of point mutations on the folding and aggregation pathways and the formation of inclusion bodies (for a review, see (King et al. 1996)). These point mutations were mainly temperature-sensitive mutants – to this entire ensemble of knowledge accumulated in the King lab over the years, I was very fortunate to add a small contribution on second-site suppressors, mutants that alleviate the folding defects caused by temperature-sensitive mutants in P22 tailspike (Mitraki et al. 1993 ; Mitraki et al. 1991 ).
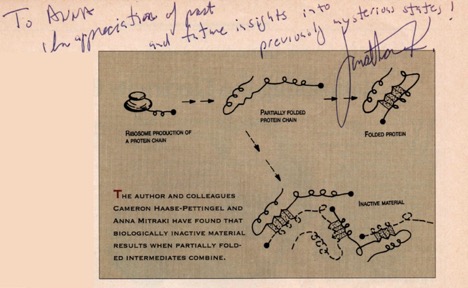
Protein aggregation is not only encountered in laboratory set-ups or during industrial production of proteins for biotechnological or therapeutic uses. In diseases such as Alzheimer’s, Parkinson’s, prion diseases etc., the so-called “amyloid” diseases, proteins are deposited in form of insoluble, fibrillar forms again with the characteristic “cross-beta” signature (Glenner 1980a, 1980b). For many decades, amyloid diseases have been the subject of intense investigation, given the pressing need for therapies of these devastating diseases. However, the general perception of protein aggregates remained the one of intractable, useless, or even dangerous entities.
However, fibrous amyloid-structured aggregates are not only associated with protein misfolding but are also exploited by Nature as structural materials, in order to convey strength and protection whenever needed, or as adhesion materials. For example, the green lacewing fly Chrysopa flava lays its eggs at the tip of fibrous proteinaceous stalks tethered on the surface of plant leaves, as a protection strategy against predation and cannibalism (Ruzicka 1997). These fibrous stalks display the cross-beta X-ray diffraction pattern (Geddes A.J. 1968). Silk moth oocytes are surrounded by chorion (eggshell) proteins with exceptional chemical and mechanical stability. Their role is to protect the developing oocyte from environmental hazards such as temperature, pressure, viruses, bacteria, etc. (Iconomidou and Hamodrakas 2008). Protein aggregates / amyloids may even play a beneficial functional role in organisms, such as yeast prions or secretory granules that sequester protein and peptide hormones (Fowler et al. 2007). The emergence of such “natural amyloids” somehow alleviated previous perceptions on their nature and their mechanical and chemical resistance stimulated an unexpected intellectual avenue for scientists, namely their study and applications as natural materials (Mitraki 2010). Protein based fibrous materials can assemble under mild aqueous conditions and are biocompatible and biodegradable. Moreover, by simple changes at the sequence level, they offer the possibility to rationally design novel biomaterials with targeted functionalities. Pioneering contributions from the late Susan Lindquist and Thomas Scheibel (Scheibel et al. 2003), and from Ehud Gazit (Reches and Gazit 2003), launched a novel distinct area of research directions, ranging from biotechnology to materials science and from bio-nanotechnology to biomedical applications (Gazit 2007; Zhang 2003). The formation of hydrogels under certain conditions is also a distinctive property of self-assembling proteins and peptides. Shuguang Zhang from MIT, starting from a serendipitous discovery, proposed such self-supporting peptide hydrogels as scaffolds for cell attachment and proliferation (Gelain, Horii, and Zhang 2007), with concrete applications in tissue engineering and drug delivery, all the way to the clinic (Gelain et al. 2021). Shuguang is another friend from the MIT years, and we still communicate frequently. He has organized five wonderful meetings that took place at the island of Crete from 1999 until 2005 (I attended all of them), that moved the field of self-assembling peptides forward. These meetings are continuing nowadays in Geneva, Switzerland. His recent recollection paper on “Self-assembling peptides: from discovery in a yeast protein to diverse uses and beyond” is really worth reading (Zhang 2020).
After leaving MIT, fibrous proteins continued to intrigue me and I started my independent research on the folding and assembly of adenovirus fiber proteins (van Raaij et al. 1999). While working on their unfolding, folding and trimerization mechanisms we (then graduate student Katerina Papanikolopoulou, also a King lab alumna, and myself) found out that short peptides (41-6 amino acids) corresponding to the fibrous shaft sequences were self-assembling into amyloid-type fibrils in the absence of a trimerization domain (Papanikolopoulou et al. 2005). Since 2004 that I joined the Faculty of the Materials Science and Technology at the University of Crete, I got interested in translating fundamental structural and folding knowledge from natural fibrous proteins into concrete integration strategies and applications in the area of fibrous bio-nano-materials. I especially focus on the study and use of amyloid-like, self-assembling peptide materials as technological objects and their integration in innovative applications (Kokotidou et al. 2018; Kokotidou et al. 2020).
In the meantime, the conceptual turns on the nature of aggregated materials continued to emerge. Inclusion bodies were recently recognized as particulate, mechanically stable entities, consisting of an amyloid-like scaffold combined with protein molecules in a quasi-native conformation (Carrio et al. 2005). Being hydrated and porous materials they can support cell attachment and proliferation (Garcia-Fruitos et al. 2009). They were additionally studied as enriched protein reservoirs for controlled protein release, as “nanopills”, or as reusable catalysts, and thus as versatile biomaterials in general (de Marco et al. 2019).
For many years, protein structural characterization techniques could not be applied to aggregated material since they were developed for soluble proteins. Structural insight was initially acquired by single crystal structures of amyloid-forming peptides from Alzheimer’s peptide, tau, amylin, etc. (Nelson et al. 2005; Sawaya et al. 2007). More recently, the development of high resolution cryo-electron microscopy techniques led to formidable structural achievements such as the determination of the Aβ Alzheimer’s peptide structure (Gremer et al. 2017), or even of tau filaments from the brains of diseased patients (Fitzpatrick et al. 2017) .
The ensemble of the aforementioned scientific advances gradually changed the perception of the scientific community on misfolding and aggregation of proteins, establishing them as processes worth studying. Moreover, they are recognized as being central to biotechnology, and attract lots of attention as they can be beneficially exploited for bio-nanomaterials design (Ke et al. 2020). Therefore, they are currently the focus of intense investigation; in the years to come, we should anticipate an expanding number of studies to appear.
For me, it has been a fascinating journey across disciplines, that started from chemistry, through biochemistry, to materials science. Jonathan taught me the importance of vision in science, to be able to look way ahead, to foresee the scientific and technological developments of the future. Some of his key statements that I always try to follow are listed below:
“Always pursue the unexpected result”
“Don’ t fall in love with your hypothesis – you need an alternative hypothesis”
“The role of a Mentor is to liberate the intellectual power of his/her colleagues”
And of course, I took the relay of Mentoring young scientists, and especially young girls and women.
Epilogue: is it possible to “unboil the egg”??
Aggregation of proteins is intimately associated with food processing, ie heating, boiling, baking, etc. Nowadays the Astbury experiments can be repeated with modern structural characterization methods, for example for commonly consumed boiled egg whites. Interestingly, these experiments also revealed a certain percentage of amyloid fibril formation, suggesting that amyloids constitute a common component of human nutrition (Monge-Morera et al. 2020). Food amyloids from abundant and cheap natural sources, even attracted recent interest for their use in technological applications, for example their integration in filters for water purification and environmental remediation (Peydayesh and Mezzenga 2021).
Nonetheless, is it possible to “unboil the egg?” i.e., to reverse aggregation / coagulation processes? Max Perutz asked this question in an article entitled “Unboiling the egg” published in “Discovery” in 1940, (Perutz 1980). The answer is yes; by using totally denaturing conditions, i.e., high concentrations of urea or guanidine hydrochloride, one could “unscramble” i.e., physically dissolve the coagulated protein to its soluble, entirely unfolded state. Then, by carefully choosing the proper renaturation conditions, it should be possible to return to the native state.
Another characteristic of the aggregation processes is that they are kinetically controlled; by careful choice of conditions, coagulation can be bypassed. Anson and Mirsky observed that if hemoglobin or albumin get denatured by acid and subsequently the equivalent amount of alkali is added at once in order to reach the neutralization point, then the protein will coagulate. They subsequently conducted the following simple experiment: an amount of alkali just insufficient to neutralize was added and the protein was left in these conditions for a few hours. Then, the remaining alkali was added; the majority of the protein remained soluble and only a small fraction coagulated. The aggregated protein has been “reversed” to recover the structural characteristics of its native form.
Recipe for Greek egg and lemon (avgolemono) sauce,
With a molecular explanation
We used to refer to this process in the King lab, when talking about aggregation processes – I promised that one day I will write it up….
This sauce is made with eggs, lemons, and juice from soup or stuffed vegetable dishes (e.g., wine or cabbage leaves, or zucchini).
For a soup, e.g., chicken soup:
– prepare the soup to the point that is still hot, but not boiling
– whisk well 2 whole eggs (yolk + white) in bowl – eggs have to be in room temperature
– add the juice of two lemons, whisking constantly
– transfer two ladles of hot soup, to the bowl, whisking well
The reason for this step is the following: you have a concentrated protein solution, in acid conditions – if you drop this into a hot solution, the egg whites will coagulate (concentrated protein solution in acid conditions + heat = perfect conditions for aggregation). The transfer of hot soup to the bowl has the purpose to dilute the protein solution and bring it to intermediate temperature. Then, transfer the contents of the bowl to the soup pot, while stirring very well. The pot has to be retired from the burner – then cover and let it rest. If you observe white chunks swimming in in the soup…. that means the egg whites coagulated, due to overheating or insufficient stirring, i.e., the experiment failed.…
An alternative way if someone wants a fluffier texture, is at the beginning to whisk very well the egg whites, almost to a “meringue” consistency, and then add the yolks, then continue as above. I personally don’t like it, since it gives a foamy and almost “soapy” texture.
If a sauce consistency is required for a dish, not a soup, then one tablespoon of cornstarch is added to the egg and lemon bowl and after adding the egg-lemon-and juice mixture to the pot, the content of the pot is not stirred, but is homogenized by gentle cyclical movements, holding the pot with the two hands. If the soup-sauce needs to be reheated, it always has to be slowly in low heat.
Enjoy!
References
Anson, M.L, Mirsky, A.E. 1931. ‘THe reversibility of protein coagulation’, J.. Phys. Chem, 35: 185-93.
Astbury, W.T., Beighton, E., Parker, K.D. . 1959. ‘The cross-beta configuration in supercontracted proteins’, Biochim. Biophys.Acta, 35: 17-25.
Carrio, M., N. Gonzalez-Montalban, A. Vera, A. Villaverde, and S. Ventura. 2005. ‘Amyloid-like properties of bacterial inclusion bodies’, Journal of Molecular Biology, 347: 1025-37.
de Marco, A., N. Ferrer-Miralles, E. Garcia-Fruitos, A. Mitraki, S. Peternel, U. Rinas, M. A. Trujillo-Roldan, N. A. Valdez-Cruz, E. Vazquez, and A. Villaverde. 2019. ‘Bacterial inclusion bodies are industrially exploitable amyloids’, Fems Microbiology Reviews, 43: 53-72.
Fitzpatrick, A. W. P., B. Falcon, S. He, A. G. Murzin, G. Murshudov, H. J. Garringer, R. A. Crowther, B. Ghetti, M. Goedert, and S. H. W. Scheres. 2017. ‘Cryo-EM structures of tau filaments from Alzheimer’s disease’, Nature, 547: 185-+.
Fowler, D. M., A. V. Koulov, W. E. Balch, and J. W. Kelly. 2007. ‘Functional amyloid – from bacteria to humans’, Trends in Biochemical Sciences, 32: 217-24.
Garcia-Fruitos, E., E. Rodriguez-Carmona, C. Diez-Gil, R. M. Ferraz, E. Vazquez, J. L. Corchero, M. Cano-Sarabia, I. Ratera, N. Ventosa, J. Veciana, and A. Villaverde. 2009. ‘Surface Cell Growth Engineering Assisted by a Novel Bacterial Nanomaterial’, Advanced Materials, 21: 4249-+.
Gazit, E. 2007. ‘Self-assembled peptide nanostructures: the design of molecular building blocks and their technological utilization’, Chemical Society Reviews, 36: 1263-69.
Geddes A.J., Parker K.D., Atkins E.D.T., Beighton A. 1968. ‘”Cross-beta” conformation in proteins’, J. Mol. Biol. , 32: 343-58.
Gelain, F., A. Horii, and S. G. Zhang. 2007. ‘Designer self-assembling peptide scaffolds for 3-D tissue cell cultures and regenerative medicine’, Macromolecular Bioscience, 7: 544-51.
Gelain, F., Z. L. Luo, M. Rioult, and S. G. Zhang. 2021. ‘Self-assembling peptide scaffolds in the clinic’, Npj Regenerative Medicine, 6: 8.
Glenner, G. G. 1980a. ‘Amyloid deposits and amyloidosis. The beta-fibrilloses (first of two parts)’, N Engl J Med, 302: 1283-92.
———. 1980b. ‘Amyloid deposits and amyloidosis: the beta-fibrilloses (second of two parts)’, N Engl J Med, 302: 1333-43.
Gremer, L., D. Scholzel, C. Schenk, E. Reinartz, J. Labahn, R. B. G. Ravelli, M. Tusche, C. Lopez-Iglesias, W. Hoyer, H. Heise, D. Willbold, and G. F. Schroder. 2017. ‘Fibril structure of amyloid-beta(1-42) by cryo-electron microscopy’, Science, 358: 116-+.
Haase-Pettingell, C. A. , and J. King. 1988 ‘Formation of aggregates from a thermolabile in vivo folding intermediate in P22 tailspike maturation. A model for inclusion body formation.’, J Biol Chem, 263: 4977-83.
Iconomidou, V. A., and S. J. Hamodrakas. 2008. ‘Natural protective amyloids’, Current Protein & Peptide Science, 9: 291-309.
Ke, P. C., R. H. Zhou, L. C. Serpell, R. Riek, T. P. J. Knowles, H. A. Lashuel, E. Gazit, I. W. Hamley, T. P. Davis, M. Fandrich, D. E. Otzen, M. R. Chapman, C. M. Dobson, D. S. Eisenberg, and R. Mezzenga. 2020. ‘Half a century of amyloids: past, present and future’, Chemical Society Reviews, 49: 5473-509.
King, J., C. HaasePettingell, A. S. Robinson, M. Speed, and A. Mitraki. 1996. ‘Thermolabile folding intermediates: Inclusion body precursors and chaperonin substrates’, Faseb Journal, 10: 57-66.
Kokotidou, C., S. V. R. Jonnalagadda, A. A. Orr, M. Seoane-Blanco, C. P. Apostolidou, M. J. van Raaij, M. Kotzabasaki, A. Chatzoudis, J. M. Jakubowski, E. Mossou, V. T. Forsyth, E. P. Mitchell, M. W. Bowler, A. L. Llamas-Saiz, P. Tamamis, and A. Mitraki. 2018. ‘A novel amyloid designable scaffold and potential inhibitor inspired by GAIIG of amyloid beta and the HIV-1 V3 loop’, Febs Letters, 592: 1777-88.
Kokotidou, C., S. V. R. Jonnalagadda, A. A. Orr, G. Vrentzos, A. Kretsovali, P. Tamamis, and A. Mitraki. 2020. ‘Designer Amyloid Cell-Penetrating Peptides for Potential Use as Gene Transfer Vehicles’, Biomolecules, 10: 31.
London, J., C. Skrzynia, and M. E. Goldberg. 1974. ‘RENATURATION OF ESCHERICHIA-COLI TRYPTOPHANASE AFTER EXPOSURE TO 8 M UREA – EVIDENCE FOR EXISTENCE OF NUCLEATION CENTERS’, European Journal of Biochemistry, 47: 409-15.
Mitraki, A. 2010. ‘PROTEIN AGGREGATION: FROM INCLUSION BODIES TO AMYLOID AND BIOMATERIALS.’ in A. McPherson (ed.), Advances in Protein Chemistry and Structural Biology, Vol 79.
Mitraki, A. , M. Danner, J. King, and R. Seckler. 1993 ‘Temperature-sensitive mutations and second-site suppressor substitutions affect folding of the P22 tailspike protein in vitro.’, J Biol Chem, 268: 20071-5.
Mitraki, A. , B. Fane, C. Haase-Pettingell, J. Sturtevant, and J. King. 1991 ‘Global suppression of protein folding defects and inclusion body formation.’, Science, 253: 54-8.
Mitraki, A., J. M. Betton, M. Desmadril, and J. M. Yon. 1987. ‘QUASI-IRREVERSIBILITY IN THE UNFOLDING-REFOLDING TRANSITION OF PHOSPHOGLYCERATE KINASE INDUCED BY GUANIDINE-HYDROCHLORIDE’, European Journal of Biochemistry, 163: 29-34.
Mitraki, A., and J. King. 1989. ‘PROTEIN FOLDING INTERMEDIATES AND INCLUSION BODY FORMATION’, Bio-Technology, 7: 690-97.
Monge-Morera, M., M. A. Lambrecht, L. J. Deleu, R. Gallardo, N. N. Louros, M. De Vleeschouwer, F. Rousseau, J. Schymkowitz, and J. A. Delcour. 2020. ‘Processing Induced Changes in Food Proteins: Amyloid Formation during Boiling of Hen Egg White’, Biomacromolecules, 21: 2218-28.
Nelson, R., M. R. Sawaya, M. Balbirnie, A. O. Madsen, C. Riekel, R. Grothe, and D. Eisenberg. 2005. ‘Structure of the cross-beta spine of amyloid-like fibrils’, Nature, 435: 773-78.
Papanikolopoulou, K., G. Schoehn, V. Forge, V. T. Forsyth, C. Riekel, J. F. Hernandez, R. W. H. Ruigrok, and A. Mitraki. 2005. ‘Amyloid fibril formation from sequences of a natural beta-structured fibrous protein, the adenovirus fiber’, Journal of Biological Chemistry, 280: 2481-90.
Perutz, M.F. . 1980. ‘”Unboiling an egg”.’ in R. Jaenicke (ed.), Protein Folding (Elsevier-North Holland Biomedical Press).
Peydayesh, M., and R. Mezzenga. 2021. ‘Protein nanofibrils for next generation sustainable water purification’, Nature Communications, 12: 17.
Reches, M., and E. Gazit. 2003. ‘Casting metal nanowires within discrete self-assembled peptide nanotubes’, Science, 300: 625-27.
Ruzicka, Z. 1997. ‘Protective role of the egg stalk in Chrysopidae (Neuroptera)’, European Journal of Entomology, 94: 111-14.
Sawaya, M. R., S. Sambashivan, R. Nelson, M. I. Ivanova, S. A. Sievers, M. I. Apostol, M. J. Thompson, M. Balbirnie, J. J. W. Wiltzius, H. T. McFarlane, A. O. Madsen, C. Riekel, and D. Eisenberg. 2007. ‘Atomic structures of amyloid cross-beta spines reveal varied steric zippers’, Nature, 447: 453-57.
Scheibel, T., R. Parthasarathy, G. Sawicki, X. M. Lin, H. Jaeger, and S. L. Lindquist. 2003. ‘Conducting nanowires built by controlled self-assembly of amyloid fibers and selective metal deposition’, Proc Natl Acad Sci U S A, 100: 4527-32.
van Raaij, M. J., A. Mitraki, G. Lavigne, and S. Cusack. 1999. ‘A triple beta-spiral in the adenovirus fibre shaft reveals a new structural motif for a fibrous protein’, Nature, 401: 935-38.
Zhang, S. G. 2003. ‘Fabrication of novel biomaterials through molecular self-assembly’, Nature Biotechnology, 21: 1171-78.
———. 2020. ‘Self-assembling peptides: From a discovery in a yeast protein to diverse uses and beyond’, Protein Science, 29: 2281-303.